Fully integrated continuous bioprocessing of recombinant proteins using mammalian cells
SUPERVISOR: Alois JUNGBAUER
PROJECT ASSIGNED TO: Daniel Paul KOMUCZKI
With the release and the embracement of recent QbD/PAT guidelines as “best practice” for process development by regulatory authorities a new trend in the biopharmaceutical industry towards integrated continuous bioprocessing (ICB) was introduced [1]. These guidelines aim to establish an in depth process understanding for the manufacturing process to be able to define on the one hand critical process parameters (CPPs – e.g. DO) and on the other hand critical quality attributes (CQAs – e.g. Glycosylation) which are affecting the protein of interest. Furthermore, to monitor and control respective CPPs and CQAs during the manufacturing process for a real-time release of the POI [2]. Important to realize is, that in contrast to conventional fed batch operations ICB requires continuous monitoring and control and, therefore, process intervention. If e.g. upon a fluctuation certain CQAs are out of acceptable ranges, process parameters or media/buffer components could be adapted to tailor the respective CQAs. For example, it has been demonstrated that by shifting of process parameters such as temperature the behaviour of the cell culture can be controlled [3]–[5]. However, also media components might be altered to simplify respective downstream unit operations [6]–[8]. Besides that, ICB offers also a higher volumetric productivity, smaller industrial footprint, flexibility, superior quality and reduced residence time of proteins.
On the downside, one of the biggest disadvantages of continuous bioprocessing technology is the high media/resin usage, complexity and the related logistic challenges [17]. Moreover, the definition of a batch from a regulatory point of view is a critical aspect especially when dealing with fluctuations during the bioprocess [16]. Thus, a major challenge for performing this transition is how to reduce manufacturing and research and development (R&D) costs. One strategy to tackle this challenge is to efficiently integrate individual unit operations into a fully continuous lab scale process and develop lab scale model system to generate the necessary data base for the required depth of process understanding. Another strategy is to leverage the information generated by the integration of the unit operation for advanced process monitoring and control. Finally, also rapid prototyping of new continuous technologies with superior characteristics especially in terms of their ease of integration into a continuous bioprocess can be used. By means of e.g. 3D-printing, offering the capacity to generate new prototypes within hours or days, rather than weeks or months, a strategy of “fail early, fast and often” and consequently reduced the R&D cost can be pursued.
Henceforth, the objective of this doctoral thesis is to first establish up-stream processes in various process modes. A (semi-) continuous downstream process based on traditional technology (chromatography) will be developed and integrated. Consequently, bottlenecks for a fully ICB e.g. media/buffer preparation will be identified and new technologies will be explored to alleviate those bottlenecks.
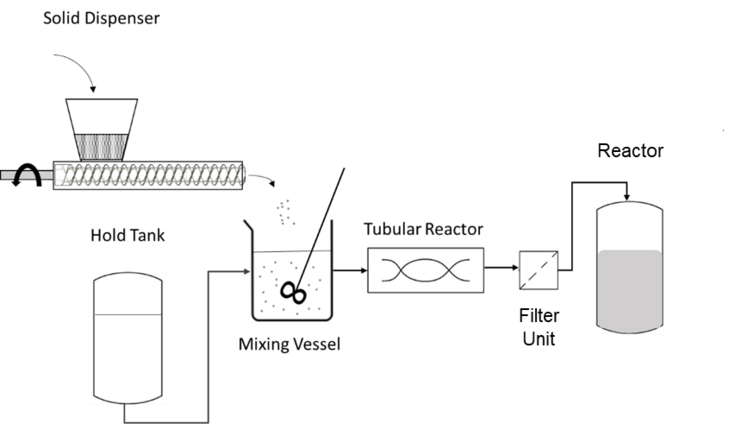
Figure 1 Development of the Dissolvr system for the continuous reconstitution of process materials
Finally, already existing technologies will be evaluated and alternative superior technologies will be developed making full use of rapid prototyping. All packages will be performed in close collaboration with other members of the A4B consortium which thrive to establish faster (e.g. fast LC-MS) and more efficient separation tools which can be integrated as a real-time feedback loop and release for the upstream or downstream production process. This collaboration will give access to a much more in-depth characterization of CQAs and subsequently lead to a much deeper insight into CPPs. The results of this thesis will support the transition towards a fully ICB which will help to reduce manufacturing and R&D costs.
This project is developed as part of the ITN “Analytics4Biologics” (A4B), funded by the European Union under the Marie Curie- Sklodowska framework.
References:
[1] R. H. P. Cc. FRSC, “QbD and PAT: A Marriage Made in Heaven?”
[2] FDA, “Guidance for Industry PAT: A Framework for Innovative Pharmaceutical Development, Manufacuring, and Quality Assurance,” FDA Off. Doc., no. September, p. 16, 2004.
[3] E. Trummer et al., “Process parameter shifting: Part II. Biphasic cultivation - A tool for enhancing the volumetric productivity of batch processes using Epo-Fc expressing CHO cells,” Biotechnol. Bioeng., vol. 94, no. 6, pp. 1045–1052, May 2006.
[4] T. Hogiri, H. Tamashima, A. Nishizawa, and M. Okamoto, “Optimization of a pH-shift control strategy for producing monoclonal antibodies in Chinese hamster ovary cell cultures using a pH-dependent dynamic model,” J. Biosci. Bioeng., vol. 125, no. 2, pp. 245–250, 2018.
[5] M. K. F. Wolf et al., “Improved performance in mammalian cell perfusion cultures by growth inhibition,” Biotechnol. J., vol. 1700722, p. 1700722, 2018.
[6] M. M. Nasr et al., “Regulatory Perspectives on Continuous Pharmaceutical Manufacturing: Moving From Theory to Practice: September 26-27, 2016, International Symposium on the Continuous Manufacturing of Pharmaceuticals,” J. Pharm. Sci., vol. 106, no. 11, pp. 3199–3206, 2017.
[7] J. M. Bielser, M. Wolf, J. Souquet, H. Broly, and M. Morbidelli, “Perfusion mammalian cell culture for recombinant protein manufacturing – A critical review,” Biotechnol. Adv., vol. 36, no. 4, pp. 1328–1340, 2018.